A Tale of Two Atoms
The kingdom of matter stores its treasures on many levels. Until recently, we thought there was only one. We had no idea there were others.
When we strike a match, a chemical reaction liberates energy stored in the molecules. Old chemical bonds break and new ones are forged. Now, the adjacent molecules begin to move faster and the temperature increases. Soon, the process becomes self-propagated, a kind of chain reaction.

But there is a deeper level of matter that houses another kind of energy. Inside the heart of the atom, its nucleus. This hidden treasure was forged billions of years ago in distant stellar furnaces. Long before Earth was formed. It’s what powers the stars. Wresting this knowledge from nature is a cosmic rite of passage. The beings of any possible world clever enough to travel this deep into nature’s labyrinth better take care. The secret of starlight is nothing to fool with. Like fire, it can bring a civilization to life and it can burn it to the ground.
What is an atom?
What are atoms made of? How are they joined together? How could something as small as an atom contain so much power? Where do atoms come from?
The same place we do. When we seek the origin of atoms, we are searching for our beginnings. This quest takes us to the depths of space and time.
Long ago, before there was an Earth, there was a wisp of cold thin gas. The gas was made of the simplest atoms. And they were gravitationally attracted to one another. So, the cloud grew. The atoms contained small but heavy particles in their nuclei. The hydrogen had protons, the helium had neutrons as well. They both had a skittering veil of electrons in orbit around them. The atoms in the interior of the cloud moved ever faster as gravity pulled them ever closer together. Until the whole thing collapsed in on itself. This collapse raised the temperature so high, that the cloud became a natural fusion reactor.
In other words, a star.
Atoms operating according to the laws of physics met and fused in the unbroken darkness.
And then there was light.
In this froth of elementary particles, the nucleus of one of the atoms, a helium atom, was formed.
After billions of years, the star is now elderly. Having converted all of its available hydrogen fuel to helium. Now that it’s time for the star to die, it resumes the turning inward of its infancy. Our helium atom joined with two others to become one of our heroes, a carbon atom. That’s what happens in the hearts of stars. Soon, our carbon atom will tumble out of this red giant star into the interstellar ocean of space.
Meanwhile, in another part of the galaxy. Similar processes were unfolding as stars were born and died. The other atom of our tale was formed in the heart of this dying star. In the catastrophic process of going supernova, 226 protons and neutrons became fused to a carbon atom. Turning it into a uranium atom.
As chance would have it, after wandering the vast Milky Way galaxy, our two atoms both happened on the fiery birth of a small solar system.
Ours.
Our carbon atom has travelled far to become part of a small planet. After billions of years, it joined an extremely complex molecule, which has the peculiar property of making virtually identical copies of itself. The carbon atom plays its tiny role in the origin of life. Through all its incarnations, our carbon atom has had no self-awareness. No free will. It is merely an extremely minor cog in some vast cosmic machinery, working in accord with the laws of nature.
And that other atom? The uranium atom made in the supernova? What has become of it?
/https://tf-cmsv2-smithsonianmag-media.s3.amazonaws.com/filer/7e/59/7e593e43-c61b-4635-9fac-8a6cd1a36ed3/accretion-nature.jpg)
Our world was born in fire. And this tiny atom was drawn to it. Maybe it rode the explosive wave of a supernova. Or perhaps, it was attracted by the gravity of our sun and pulled down deeper and deeper into the interior, which was even more of a hell.
The Earth’s surface soon cooled, but the interior remained molten. The magma slowly circulating and our uranium atom found itself carried over the ages, from the deep interior, back up to the surface. Despite the high temperatures and pressures deep within the Earth, our atom’s integrity was never threatened. Atoms are small, old, hard and durable.
Everything is made of atoms, including us
Until the last years of the 19th century, we didn’t know about the frenzied activity inside the atom. And this is where our two atoms from opposite ends of the Milky Way galaxy finally met. It happened in Paris.
Our carbon atom became part of the retina of one of the world’s greatest scientists. This was just a few years after the discovery of X-rays.

The dull brown ore, still mixed with pine needles, came from the part of Eastern Europe that is now the Czech Republic. But this material was very rare. And even to distil a tiny amount of it required the most lengthy and labour-intensive efforts.
We lived in our single occupation, as in a dream.
Marie Curie
They worked under the worst possible conditions to purify the ore into a mineral called pitchblende, which was 50 to 80% uranium. This was quite an achievement, but Marie and Pierre were hunting for something far more rare. It took them three years to process tons of ore. To isolate a mere tenth of a gram of a substance she named radium.
Marie and Pierre had discovered a completely new element.
The Curies showed that the radium was entirely unaffected by extreme temperatures. That was strange. Most things subjected to such intense heat would change drastically. And, there was something else. It spontaneously emitted energy. Not through chemical reactions, but through some unknown mechanism. Marie Curie called this new phenomenon “radioactivity”. She and Pierre calculated the energy that spontaneously flowed from a lump of radium would be much greater than burning the same amount of coal. Radioactivity, to their astonishment, was millions of times more potent than chemical energy – the difference between liberating the energy that resides in molecules and the far greater power stored deeper down.

The bottles, tubes and flasks of pitchblende that they had refined, left a residue of radium particles. They were so potent, that they lit up the lab at night. As Marie wrote years later, “They were like Earthly stars, these glowing tubes in that poor rough shack.” Marie leapt to the correct conclusion that the luminescence was due to something happening inside the nuclei of radioactive atoms.
A World Set Free
For thousands of years, it had been thought that atoms were the smallest unit of matter. Curie’s earthly stars were evidence that within the atom was a possible world where even smaller particles were interacting. A hundred years after this magical night, Marie Curie’s cookbooks still glowed with the exquisite radioactivity she had discovered.

A writer, H. G. Wells was a genius at turning the new revelations of science into stories that captivated the world. And foreseeing as no one else, their gravest consequences. The writer H.G. Wells, who first imagined time machines and alien invasions had a nightmare of a future world where atoms were weaponized. In his book called The World Set Free written in 1913, he coined the phrase atomic bombs. And loosed them on helpless civilian populations. He set his vision of a nuclear war between England and Germany in the impossibly distant future of the 1950’s.
In 1933, the Hungarian physicist, Leo Szilard, was contemplating becoming a biologist. He read Wells’ novel and it started him thinking. Szilard knew that atoms are made of protons and neutrons on the inside. And a skittering veil of electrons on the outside. Suddenly, waiting for a traffic light to change at an intersection in London, he was struck by the thought, that if he could find a sufficiently large amount of an element that would emit two neutrons when it absorbed one, it would sustain a nuclear chain reaction. Two would produce four, four would produce eight and so forth. Until enormous amounts of energy in the nucleus itself could be liberated. Not a chemical reaction, but a nuclear one.
This was the moment our world changed. Leo Szilard also knew the power of exponentials and if a neutron chain reaction could be triggered down there in the world of the atom’s nucleus, then something like Wells’ imaginary atomic bomb might be possible. He shuddered at the thought of this destructive capability.
But this was just the latest development on a continuum of violence that began long long before.
War, a History
50,000 years ago, all humans were roving bands of hunter-gatherers. They communicated over limited areas by calling to one another. That is, at the speed of sound. Around 1,235 kilometres per hour. But over longer distances, they could communicate only as fast as they could run.
Around 12,000 years ago, about the same time as the invention of agriculture, they developed the power to kill at a longer distance. The kill radius expanded to the arc of an arrow launched by a bow. And they could kill one person with a single arrow. Our ancestors were not particularly warlike because there were so few people and so much room back then that moving on was preferable to armed conflict. Their weapons were used almost entirely for hunting. Their identification horizon was likely small. Only with the other members of their band of 50 or 100 people. But their time horizon took a giant leap. They worked long and hard planting crops in the here and now so that several months later, they could harvest them. They postponed present gratification for later advantage. They began to plan for the future.
By about 2,500 years ago, there was a new kind of war. The conquered territories of Alexander stretched from Macedonia to the Indus Valley. There were now many on planet Earth who owed allegiance to groups composed of millions. Over long distances, the maximum speed of both communication and transportation was the speed of the sail and the horse. Archidamus III, King of Sparta, was famed for his unflinching courage. He relished taking part in hand-to-hand combat with the enemy. It is said that when he first saw a projectile hurled by a Balista, he cried out in anguish. “Oh, Hercules! The valour of man is lost!”. Both the kill range and the kill ratio had increased exponentially. Now, ten corpses lay where one would have been. And the soldier who released the lever on the siege engine never even saw their faces. He remained far removed from the carnage on the other side of the city wall.
Today, the maximum speed of transportation is the escape velocity from Earth. 40,000 kilometres per hour. The speed of communication is the speed of light. The identification horizons have also expanded enormously. For some, it’s a billion or more. For others, it’s our whole species. And for a few, it’s all living things. The kill radius, in the worst-case scenario, is now our global civilization.
How did we get here?
Well, it was the result of a deadly embrace between science and state. And there was one scientist for whom no amount of destructive power was enough.
It’s hard to pinpoint the precise moment when the first nuclear war began. Some might trace it back to that arrow sailing over the treetops. Others might say it started much later, with three messages.
In 1939 on Adolf Hitler’s birthday, one of his brightest young scientists, Paul Harteck, had a special gift in mind for his Führer. Harteck wrote a letter to the Nazi war office, he wished to inform them that the latest developments in nuclear physics would make it possible to produce an explosive exponentially more powerful than conventional weapons. He was trying to give an atomic bomb to Adolf Hitler. But Hitler would never get his hands on a nuclear weapon, he had murdered, imprisoned or exiled many of the great physicists in his territories. Those who happened to be Jews or liberals and many who were both.
Exactly a month before the war began, Leo Szilard made a pilgrimage to the house Albert Einstein was renting on Long Island in New York. The physicist who usually chauffeured Leo Szilard on trips out of Manhattan was unavailable that August day in 1939. So, Szilard enlisted the services of a fellow Hungarian emigrate, a young scientist named Edward Teller. Persecution in Budapest sent Teller and his family to take refuge in Munich, where he lost his right foot in a traffic accident. In the early 1930s, Teller and his family were forced to flee once again.
Just as Harteck felt it his duty to inform Hitler. Szilard wanted the US President, Franklin Roosevelt, to know the awesome power of such a weapon. There was no scientist on Earth whose prestige and influence was comparable to Einstein’s. Einstein’s nightmare was imagining Hitler with a nuclear weapon at his disposal. But what would be the long-term consequences of this dangerous new knowledge? Which, once unleashed, could never be taken back. Einstein would take no role in the U.S. effort to build the atomic bomb, which became known as “The Manhattan Project.” But he did alert the then-U.S. President. Franklin Roosevelt, to the potential use of atomic nuclei in warfare. After the war was over, he told a reporter that if he had known the Germans would fail in developing an atomic bomb, he never would have signed the letter. But Edward Teller had no such ambivalence. He couldn’t wait to get started on weaponizing the atom.
The Russian physicist, G.N. Flyorov had tried for years to alert his leader, Joseph Stalin, to the possible military applications of a nuclear chain reaction. However, the Soviet Union was under siege by the Germans. And an atom bomb project was likely to take years to complete. With their backs against the wall, it seemed too impractical to even think about. In 1942, Flyorov had published a scientific paper on nuclear physics. Now, he was excited to see what the eminent physicists in Europe and the United States had to say about it. Flyorov was puzzled. None of the physicists of the International Scientific Community thought his paper worthy of comment.
At first, he was hurt, but then he realized what was really happening. American and German scientific journals were being scrubbed of any nuclear physics papers as both nations secretly worked on building the bomb. It was this absence of published data, the dogs that did not bark, that moved Flyorov to re-double his efforts to convince Stalin to start his own nuclear weapons program.
In all three cases, it was the scientists, not the generals or the arms dealers, who informed their leaders that a huge increase in the kill ratio was possible.
The Manhattan Project
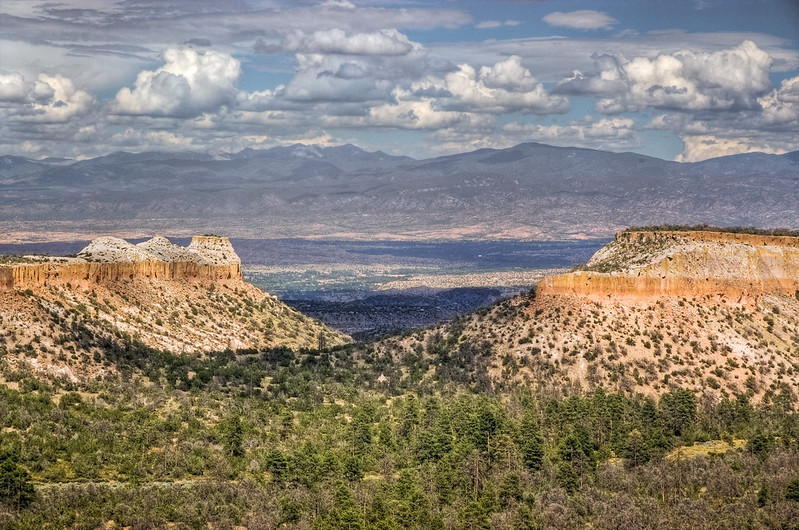
But for Edward Teller, an atomic bomb wasn’t big enough. He dreamed of even greater lethality. A weapon in which the atomic bomb was nothing more than a match to light a fuse to the nucleus. A thermal nuclear weapon. What Teller affectionately called, the super.
If Edward Teller had a polar opposite in the scientific community, it would have been Joseph Rotblat. Rotblat was born in Warsaw to a wealthy family, who like Teller’s, had lost everything. In the summer of 1939, just before the Nazis invaded, he was invited to England to take a research position at the University of Liverpool. At the last minute before his departure, his beloved wife, Tola had an emergency appendectomy. She was forced to remain behind until she was well enough to travel. Tola insisted that Joseph go on ahead to prepare their new home. It would just be a matter of weeks, she told him.
The challenge to the Manhattan Project team was to find a chemical fuse that would light the nuclear chain reaction, first imagined by Leo Szilard in London. The scientists and engineers told themselves that they would be averting a grave danger by building a bomb of unprecedented destructive power. Their government could be trusted. They would never use such a weapon in an act of aggression, not like those other governments. These atomic scientists were the first to see building nuclear weapons as a deterrent to using them. The fear of Hitler with an atomic bomb was the driving rationale for the Manhattan Project.
And yet, when Germany surrendered and Hitler was no more, of the thousands of scientists who worked on the bomb, only one resigned. It was Joe Rotblat. In the years that followed, whenever he was asked about his decision, he always rejected any suggestion that he had done so out of moral superiority. He would just smile and say, the truth was that he desperately missed his wife, who had been prevented from leaving Warsaw and lost to him in the chaos of the war. With its end in Europe came his chance to go and search for her. But, he never found her. Except as a name on a list of the dead. Tola had perished in the Holocaust, exterminated at the Belzec concentration camp. Although he lived another 60 years, Rotblat never remarried.
Of the three nations that pursued wartime research into building the bomb, only the U.S. succeeded before the war’s end. And historians believe that was because America had taken in so many immigrants. Of the leading figures in the Manhattan Project, only two were native-born. Only one got his PhD in the U.S. Atomic bombs were dropped on the Japanese cities of Hiroshima and Nagasaki, ending the Second World War. Two months later, President Truman invited Oppenheimer for congratulations in the Oval Office. But to Truman’s dismay, Oppenheimer was in no mood to celebrate.
Less than four years later, the Russians exploded their atomic bomb. And shortly after, both nations went on to create thermonuclear hydrogen bombs. The nuclear arms race begun by those three letters from scientists was off to a terrifying start. After the war, Teller’s dreams of greater and greater killing power were to come true. In the early 1950s, when the Communist witch hunts began in the United States, he was perfectly happy to hint that Robert Oppenheimer, his former boss, who had brilliantly run the Manhattan Project, should be stripped of his security clearance, thereby ruining Oppenheimer’s career.
Despite dramatic reductions in nuclear arsenals, the spectre of nuclear war haunts us still. How can we sleep so soundly in the shadow of a smoking volcano?
A Tale of Two Atoms
We’re back on the trail of one of our two atoms. The uranium atom. A uranium atom is inherently unstable. Sooner or later, it decays. A particle from its nucleus breaks away, transforming the uranium atom into an entirely different element. Thorium. Subatomic particles move like bullets through the fine structure of life. Shearing electrons from their molecules. This is how ionizing radiation affects living things. Those chromosomes never had a chance. This is why atomic weapons are so much more dangerous than conventional ones. Ionizing radiation is all around us and even inside us. At low levels, it poses no threat. But at higher levels, it’s a different story.
In the near term, exposure to lethal levels of radiation can cause a runaway reaction in the cell that makes it multiply exponentially. Cancer. But its power to harm can also echo down the corridors of time. When radiation tears into the chromosomes of the butterfly, it leaves a trail of destruction in its wake that changes the destiny of the butterfly’s unborn offspring. A mutation in its genes We have a lot in common with butterflies. Any change in the DNA architecture will be copied over and over again in succeeding generations. The damage is passed on. Vandalizing our future.
We are made of atoms that were born in stars thousands of light years away in space and billions of years ago in time. The search for our origins has carried us far from our epoch in our world. We are star-stuff, deeply connected with the rest of the universe. The matter we are made of was generated in cosmic fire. And now, we, ambulatory collections of seven billion billion billion atoms intricately assembled over aeons have devised a means to tap that cosmic fire, hidden in the heart of matter.
We cannot unlearn this knowledge. And tragically, insanity runs in our family.
The letters that the scientists wrote to begin this nightmare were followed by another. This one, a letter to the planet, stating that this new understanding of physics demanded a new way of thinking:
Shall we choose death because we cannot forget our quarrels? We appeal as human beings to human beings, remember your humanity and forget the rest.
And what of our other atom? The carbon atom? It’s inside one of you.